PFAS Monitored Retention (PMR) and PFAS Enhanced Retention (PER)
Perfluoroalkyl and Polyfluoroalkyl Substances (PFAS) Monitored Retention (PMR) is an approach for managing PFAS-affected sites that relies on natural retention processes that can lessen the migration and maximum concentrations of many PFAS at impacted sites. Because PFAS have not yet been shown to degrade to harmless end products by natural abiotic or biological actions, these retention processes (e.g., sorption, matrix diffusion) are expected to be the primary factors that dictate how PFAS behave and move in the environment [1][2][3][4][5][6][7], and they form the basis for PMR. The PMR concept has been incorporated into a framework (the “PMR Framework”) that can help users determine the suitability of PMR at a specific site or identify the highest priorities among a portfolio of PFAS-affected groundwater sites. The Framework provides guidance on collecting different types of data (lines of evidence) to support a PMR evaluation. It also describes enhanced retention strategies known as PFAS Enhanced Retention (PER) that may be applicable for management of sites where natural retention is insufficient on its own to protect downgradient receptors.
Related Article(s):
- Monitored Natural Attenuation (MNA)
- Perfluoroalkyl and Polyfluoroalkyl Substances (PFAS)
- PFAS Transport and Fate
Contributor(s): Dr. David Adamson, P.E., Dr. Charles Newell, P.E. and Dr. Hans Stroo
Key Resource(s):
- Framework for Evaluating PFAS Monitored Retention at PFAS Groundwater Sites
- Monitored Natural Attenuation to Manage PFAS Impacts to Groundwater: Scientific Basis[2]
- Monitored natural attenuation to manage PFAS impacts to groundwater: Potential guidelines[3]
- Enhanced Attenuation (EA) Processes to Manage PFAS Plumes in Groundwater[8]
Introduction
Groundwater sites contaminated with PFAS are difficult to investigate and remediate due to strict cleanup goals, lack of natural degradation mechanisms for some PFAS, and the high mobility and persistence of several PFAS. Several factors contribute to the urgency of developing cost-effective strategies to manage PFAS sites:
- The number of groundwater sites with PFAS that will require some type of management could be in the tens of thousands[8][9]. Recent estimates suggest that there are 50,000 to 60,000 potential PFAS sites in the U.S[10][11].
- No technologies currently exist that are capable of effectively and efficiently destroying PFAS in situ. Furthermore, the remediation industry is unlikely to be able to practically manage the large number of PFAS groundwater plumes using the only two technologies that are currently viable, groundwater pump and treat and in situ sorbents[8].
- Despite a lack of natural processes that permanently sequester PFAS, there are natural processes can potentially retain PFAS in the subsurface for extended periods, limiting contaminant migration.
- Consequently, retention could be an important factor in reducing the near-term risk associated with PFAS groundwater plumes at some sites and open up approaches for managing PFAS plumes based on retention[2][3][8].
- Even after active remediation, it is likely that low residual concentrations of PFAS will remain in soils and groundwater, and these may also be effectively controlled by retention.
As a result, understanding and potentially relying on processes that reduce PFAS migration rates and mass discharge rates is of considerable interest to site managers. This includes a variety of chemical and geochemical retention processes that have been incorporated into the PFAS Monitored Retention (PMR) approach that site owners, their consultants, and environmental regulators can use to prioritize and manage their portfolios of PFAS sites.
PMR versus MNA
PMR is a similar concept to monitored natural attenuation (MNA), and the term has recently been adopted in place of MNA to avoid potential confusion with destructive and/or permanent attenuation processes that are part of the MNA strategies for other constituents of concern (COCs). However, many of the processes remain the same, and they are expected to reduce PFAS concentrations and mass discharge during transport from source areas.
The use of management based remedial approaches like MNA to control PFAS plumes poses several challenges, including that traditional MNA applications typically deal with petroleum hydrocarbons and chlorinated solvents, which can naturally break down to harmless end products at many sites. However, MNA as a remedy or site management strategy has been approved by regulators for some non-degrading metals, metalloids, and radionuclides (e.g., chromium, arsenic, and uranium) if the local geochemical conditions can sequester or immobilize these contaminants. This implies that even if some PFAS do not naturally degrade, passive management strategies may be feasible for PFAS plumes in groundwater because PFAS retention processes can help reduce their concentrations. Figure 1 shows how PMR fits into the development timeline for MNA-type approaches for different contaminant classes.
A key concept of PMR is that retention processes can provide a credible scientific basis for attenuation of PFAS concentrations or mass discharge over time (or distance) at some PFAS sites. The concept of using these processes in a manner similar to MNA to manage PFAS sites was first proposed by Newell et al.[2][3], which identified several retention processes, including various sorption mechanisms, geologic matrix diffusion, geochemical conditions that limit the biotransformation of precursors, and dispersion of migrating PFAS plumes. A subsequent paper[8] distinguished between sequestration/immobilization and retention, noting that while permanent sequestration of PFAS compounds has not been confirmed, significant long term retention processes are likely present. These papers discussed how retention processes vary between different types of specific PFAS and PFAS classes, as well as the critical differences between the terms “retention” and “sequestration” in the context of managing groundwater plumes[8]. These two concepts may be summarized as:
- Sequestration (or immobilization): The permanent trapping and isolation of a chemical in the environment in a natural or artificial storage compartment, such that the chemical does not impact potential receptors.
- Retention: The storage of a chemical in the environment so that the chemical is isolated from potential receptors for a certain amount of time.
This distinction is important because permanent sequestration processes for individual PFAS in the subsurface have yet to be established. Conversely, the MNA approach designed for metals and radionuclides uses the term immobilization as a key process for implementing MNA[12]. In the case of PMR, the focus is on retention processes that reduce the mobility and risk associated with PFAS in the subsurface.
PFAS Retention Processes
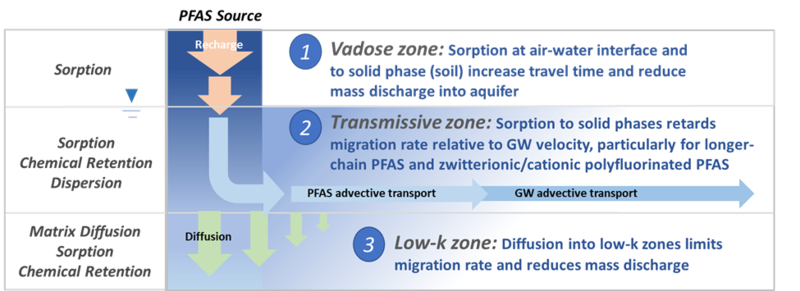
Key retention processes for PFAS in the subsurface (shown in Figure 2) include:
Sorption of PFAS to solid phases like soil particles has been well documented. It depends on mechanisms like hydrophobic sorption and electrostatic interactions which are a function of the physical-chemical properties of the PFAS and the environmental matrices[2]. In addition, air-water interfacial sorption of PFAS can be an important retention process in the unsaturated zone due to the presence of porewater and air-filled pore space in that soil compartment. In general, these can be considered “geologic retention” processes because they directly relate to interactions between PFAS and the surrounding porous media.
Matrix Diffusion refers to the diffusion of chemicals into and out of low-permeability soils (e.g., clays, silts) that are present in heterogeneous geologic settings. Previous studies with non-PFAS contaminants have shown that the mass retained in low-permeability units can serve as a persistent source of contaminants in groundwater[13]. Recent research has shown that matrix diffusion can also play a significant role in attenuating the expansion of groundwater plumes for non-degrading or highly recalcitrant compounds like perfluorooctanesulfonic acid (PFOS)[14].
Chemical Retention refers to the retention of polyfluoroalkyl substances in their “precursor” state. The mobility of these polyfluorinated precursors may be more limited because the head group increases the size of the molecule and may include positive charges that increase sorption to particles. Precursors can be transformed to perfluoroalkyl acids (PFAAs), which are generally more mobile in groundwater but not subject to further degradation under natural conditions. Therefore, precursors can be seen as "chemically retained" PFAS mass. This distinct characteristic means that biotransformation acts as a sink for solvents and hydrocarbons but as a source of PFAAs at PFAS sites. These PFAS precursor transformations appear to occur more rapidly through aerobic biological processes (or after introducing chemical oxidants to remediate other constituents)[15], such that chemical retention is more likely under anaerobic conditions.
Dispersion describes the process by which a groundwater plume spreads out as it moves through porous media. While this process can contribute to natural attenuation of contaminants over time, recent advances in modeling dispersion have shown that it may not be as significant for groundwater plumes as previously assumed[16]. When a plume reaches a certain length (which can be hundreds or thousands of meters), the weak natural mixing that happens because of transverse dispersion (the dispersion perpendicular to the primary direction of contaminant transport) may limit further movement of the plume.
Because PFAS is a class of compounds, it is important to note that not all PFAS are retained identically. For instance, longer-chained PFAS are generally less mobile and more strongly retained than shorter-chain PFAS. This same relationship applies to various other sorption processes. Perfluorinated sulfonic acids (PFSAs) tend to be more strongly retained than perfluorinated carboxylic acids (PFCAs) of equivalent chain length. As described above, polyfluorinated precursors are generally more strongly retained than their PFAA transformation products due to their size and/or charged head groups that promote sorption.
Benefits
Retention processes have the potential to reduce the rate that PFAS plumes advance relative to groundwater flow, which can increase the time before receptors are affected. In addition, some retention processes render the plume susceptible to "hysteretic" retention processes[17][18][19][20], such as when desorption occurs at a slower rate than sorption or when matrix diffusion loading occurs more rapidly than back-diffusion. At sites where retention capacity is substantial, hysteretic processes attenuate the mass discharge of the plume by reducing the peak mass discharge and concentration (“peak dampening”), extending the plume discharge over a longer timeframe. Finally, at some sites, retention processes coupled with dispersion could be substantial enough to stabilize the PFAS plume, thereby hindering further expansion. If the source of the plume has been removed or isolated, the PFAS plume will eventually diminish under such conditions.
PMR can offer several additional benefits for both site managers, regulatory authorities, and the public, such as:
- Reducing the environmental footprint and impacts of PFAS management
- Reducing the costs and complexities of PFAS management
- Providing time and flexibility for future decision making at sites with significant retention
PMR/PER Framework
Lines of Evidence
For PFAS sites, the three Lines of Evidence (LOE) originally developed for MNA of other COCs (see Monitored Natural Attenuation (MNA))[21] have been heavily modified to develop lines of evidence as part of the PMR Framework. Because there are no known processes that destroy the fully fluorinated PFAA class of PFAS in the environment, the demonstrated mass loss requirement for MNA Line of Evidence 1 has been modified to demonstrate that PFAS plumes have enough retention to pose no near-term risk to receptors. Therefore, for PMR projects:
The First Line of Evidence is to determine where a site fits into a PFAS Plume Management Scenario based on two key retention metrics: mass discharge (Line of Evidence-1A) and travel time to potential receptors (Line of Evidence-1B) (Figure 3). Sites that are most amenable for PMR will be sites with 1) relatively lower PFAS Mass Discharge; and 2) stable or shrinking PFAS plumes, or alternatively long travel times before the closest receptors are impacted (e.g., 10 years or more). Note that ongoing groundwater monitoring (and likely land use controls) would be required as an additional safety factor to ensure that receptors are protected.
The Second Line of Evidence involves collecting site specific field data that help establish that specific retention processes are active at the site. This includes documenting matrix diffusion (e.g., significant geologic heterogeneity) in unconsolidated settings by collecting samples in low-permeability zones (e.g., silts, clays) and analyzing these for PFAS and soil organic carbon. The PFAS concentrations or mass can be compared to the concentrations or mass of PFAS in soils in the transmissive zones containing the PFAS plume. Co-located groundwater and soil samples can be collected to demonstrate significant ongoing sorption processes in key compartments and to estimate field-based partition coefficients (Kd) to help estimate retardation factors for PFAS. For chemical retention, field sampling can be performed to establish geochemical conditions and the total mass present as precursors versus PFAAs in various portions of the site. Another option for the Second Line of Evidence includes quantifying the mass discharge of PFAS from the vadose zone that is entering groundwater. This can be attempted using direct porewater measurements (lysimeters), partitioning calculations, or leaching tests[22][23][24]. A primary goal of quantifying the mass discharge from the vadose zone is to evaluate if its contributions to groundwater are insufficient to necessitate soil cleanup.
The Third Line of Evidence uses special field measurements or modeling studies to better establish how ongoing retention processes influence PFAS transport to potential receptors.
The first two Lines of Evidence for PMR described above have been incorporated into a qualitative framework for managing PFAS sites (Figure 3) to help determine the suitability of PMR at a specific site. Establishing relevant (site specific) values for these two key retention factors is discussed below.
Evaluation Tiers
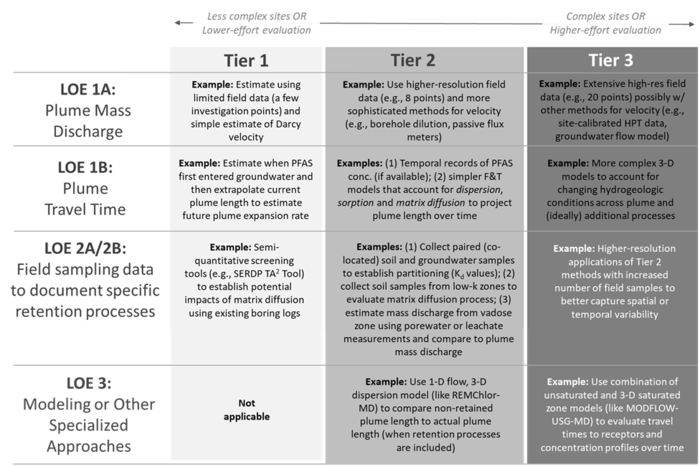
The PMR Framework relies heavily on site characterization data, with fate and transport modeling as a complementary option to support the various Lines of Evidence. The Framework contains protocols for collecting these data to support a PMR evaluation, but not all methods may be necessary at every site. Specifically, the complexity and widely varying characteristics of PFAS sites, as well as the resources that can be involved in obtaining data, necessitate differing degrees of effort for their appropriate management. Factors such as the source and plume size, proximity to receptors, and groundwater concentrations will markedly influence the required actions. Consequently, a stratified, three-tiered approach (Tier 1, Tier 2, Tier 3) has been proposed for potential PMR evaluation, with the goal of implementing the appropriate level of investigational effort and complexity for a particular site:
Tier 1 Evaluation: A limited number of conventional groundwater samples and soil samples may be sufficient for assessing PMR efficacy at less complex PFAS sites and/or sites with no or low immediate risk. A Tier 1 evaluation may also be appropriate as an initial screening step in evaluating a portfolio of sites as a basis for making decisions about performing further (higher tier) investigations. The data gathered for the First and Second Lines of Evidence can be based on this limited sampling. Simple data analysis techniques, such as calculating the PFAS plume advancement rate and then extrapolating from the current PFAS plume length to estimate the length of the plume in the future, can be employed to get a conservative estimate of potential plume growth.
Tier 2 Evaluation: A more detailed evaluation may be necessary at sites with increased complexity and risk, or where the existing data are limited or less certain. In these cases, a more rigorous documentation of the First and Second Lines of Evidence should be undertaken to better understand the critical site specific retention processes. This could also include a basic Tier 2 groundwater modeling study that incorporates matrix diffusion effects within a straightforward groundwater flow regime (e.g., the ESTCP REMChlor-MD model or the ESTCP REMFluor model that is now being developed as part of ESTCP project ER24-8200). The goal in this case would be to estimate how much further plume expansion might be expected in the future.
Tier 3 Evaluation: The most complex sites with more immediate risk may require high resolution site characterization techniques akin to those utilized by Adamson et al.[4][25]. These techniques augment the First and Second LOEs, enhancing comprehension of mass distribution across different environmental compartments, the extent of chemical retention onsite, and the mass flux relative to the distance from the source. A sophisticated three dimensional model that can better manage complex groundwater flow patterns and geological heterogeneity could be considered at this tier. This is because conventional finite-difference groundwater flow and transport models exhibit substantial limitations when modeling matrix diffusion[26].
There is some subjectivity to determining whether a site is a Tier 1, Tier 2, or Tier 3 based on risk and/or complexity, but the key metrics of mass discharge and travel time to receptors can play a role in determining which Tier is initially best suited for a particular site. Figure 4 shows examples of how the Tiered approach can be used to collect data for the various Lines of Evidence for PMR.
Applications and Relevance to Managing PFAS-Affected Sites
PMR can be used for different purposes. It may have applicability as a sole remedy at low-risk sites, but it could also help control low levels of remaining contamination after active treatment. It may also serve as a temporary remedy at sites with no proximate receptors, giving time for the development of more cost effective technologies than the current pump-and-treat methods. Finally, it can be part of a treatment train at more complex sites where risk-based approaches are acceptable. The use of PMR as part of a site remedy would be expected to result in cost savings, particularly given the large number of PFAS sites and the high costs of current PFAS remedies[9][27][28].
PMR is most suitable for sites where: 1) the natural retention processes alone can protect receptors in the short-term, allowing PMR to be used as a temporary remediation measure for several years or decades; 2) very low concentrations (or mass discharge) of PFAS are present and/or PFAS plumes are stable, making them suitable for using PMR as the only remedy; or 3) as a polishing technology for low levels of remaining contamination that will often be present after active remediation. Because many PFAS plumes may be expanding, there may be some reluctance to use PMR as the final remedy under the CERCLA regulatory program. If so, sites could apply the PMR Framework to determine if PMR could be used in an Interim Record of Decision (ROD) with a Contingency Remedy; as a polishing step after initial remediation step(s); within a Groundwater Management Zone where some plume expansion is allowed; or as a resource allocation tool for PFAS site characterization.
PMR as a sole remedy is more suitable for sites with small, low-level sources that release PFAS slowly into groundwater that moves slowly or has a long travel time to important receptors and a high capacity to retain PFAS mass in the chemical and geological environment. Sites where PMR is part of a treatment train or a risk-based approach may have different characteristics, but a long travel time to a receptor is likely to be a key factor in any case. Also, PFAS retention may be higher in hydrogeologic settings that are not uniform because of the diffusion of PFAS into the matrix. Sites where most of the PFAS mass is in the form of polyfluorinated precursors (determined by using the Total Oxidizable Precursor (TOP) Assay or non-target analyses such as Total Organic Fluorine) may also be more favorable for PMR because these precursors tend to move slower than PFAAs. Sites where the groundwater is mostly anaerobic are also more favorable for PMR because these conditions should slow down the conversion of precursors to PFAAs.
Site specific PMR evaluations can be performed using numerical criteria for the two primary Lines of Evidence that are developed based on the expected constraints at the site. These can then be combined with other site specific data to evaluate the secondary and tertiary Lines of Evidence for retention. For example, if the nearest downgradient receptor was a drinking water supply, then the acceptable mass discharge for PMR would be based on not causing an exceedance of any applicable drinking water criteria (e.g., PFOS proposed MCL of 4 ng/L). This can be back-calculated by knowing the mixing ratio in a receiving stream (in the case of groundwater discharge to a surface water source of drinking water) or the pumping rate of a production well (in the case of groundwater source of drinking water with compliance measured at the wellhead)[29]. The travel time of key PFAS to the receptor would also be calculated and then compared to acceptable site specific values (e.g., travel times of >10 years).
Initial estimates of these metrics may be uncertain given the limitations in available site data to fully delineate the plume boundaries and/or the relevant concentrations for mass discharge. The level of uncertainty should help guide how much additional characterization data might be needed at a site to improve the estimates. For a simple Tier 1 evaluation, the mass discharge at the source is used for evaluating Line of Evidence 1A because it is typically conservative (i.e., it assumes that the highest concentrations at the site will eventually reach the downgradient receptor). A site with limited existing data might not seem favorable for PMR based on this type of planning-level evaluation, but this outcome may signal the need to collect additional data to reduce uncertainty. This could motivate moving to a Tier 2 or Tier 3 evaluation, where the assumption is that additional data will provide a better basis for projecting travel time and mass discharge (as well as helping to establish if retention processes are active).
An alternative application of the Framework is for multi-site evaluations where generic criteria for Line of Evidence 1A (plume mass discharge) and Line of Evidence 1B (travel time to receptor) are developed and then applied to a wide range of sites. These criteria are used to build a generic framework, and then individual sites are plotted on the Framework to help visualize priorities for cleanup (i.e., those that fall in the upper right of Figure 3).
PFAS Enhanced Retention (PER)
PFAS Enhanced Retention (PER) approaches are designed to help manage those sites where natural retention mechanisms alone are insufficient to ensure that the primary Lines of Evidence (low PFAS mass discharge, long PFAS travel time to nearest receptor) for PFAS Monitored Retention (PMR) can be met. At these sites, one of more of the key PFAS retention mechanisms may be active, but site-specific data suggest that some type of intervention will be necessary (now or in the near future). These sites represent a higher priority for an action within the context of the PMR Framework (Figure 5, Panel A), but the conditions do not require the immediate implementation of an active source remediation (e.g., the installation of a pump-and-treat system, or a point-of-use treatment to protect downgradient receptors. For PER, the overall goal is to reduce loading from the source and/or increase the retention capacity within the PFAS source area or plume (Figure 5, Panel B).
For PFAS sites, multiple different PER approaches were proposed by Newell et al.[8], including several that are currently in use or in active development including: (1) injecting particulate sorbents such as carbon into the subsurface to enhance retention (e.g. Permeable Sorption Barrier); (2) capping to retain PFAS in the vadose zone by preventing infiltration of water from the surface which could otherwise cause leaching of PFAS into groundwater; (3) gas sparging in unconfined aquifers to potentially concentrate and retain PFAS in the capillary fringe above the water table; (4) retaining PFAS via salting out processes in coastal environments where a fresh water plume may mix with saline groundwater causing increased adsorption of PFAS to solids; (5) emplacing particulate sorbents and/or ion exchange resins and/or coagulants in closely spaced permeable columns or trenches using geotechnical equipment; (6) injection of emulsified vegetable oil (EVO) to promote sorption, exhaust available oxygen, and create an anaerobic environment which retards the aerobic biodegradation of long-chain precursors into the short-chain PFAAs which are associated with greater mobility and health risks.
Implementation Issues
PMR and PER can be evaluated as a remediation technology for PFAS-impacted sites under any regulatory jurisdiction. By adapting the Framework to the specific requirements of each site, project managers can develop targeted and effective strategies for managing PFAS contamination. In terms of its broader applications within the CERCLA process, the concept of a PFAS plume expanding indefinitely might limit the use of PMR as a single final remedy in a Final Record of Decision. Mass retention of PFAS (as opposed to remediation that involves permanent removal, destruction, or sequestration of the PFAS mass) may be less favored by some regulatory authorities. However, the PMR Framework can be utilized in other ways to help manage and remediate PFAS in groundwater (as described in the Applications and Relevance to Managing PFAS-Affected Sites section above) PMR requires long-term monitoring and maintenance to ensure its effectiveness and reliability. Sites may also need land use restrictions or institutional controls to prevent exposure and further mass loading. PMR involves the long-term storage of PFAS-affected materials onsite, which may represent uncertainties and liabilities for site managers. PMR may also require increased engagement and risk communication with the stakeholders and the public, to explain the rationale, effectiveness, impacts, and future plans of PMR. It is important to communicate with the stakeholders and the public in a transparent and proactive manner, and to address their concerns and questions adequately. The implementation of PMR (or PER) as a management strategy may require that a site have a Plume Assimilative Capacity Zone (PACZ) downgradient of the source to accommodate plume expansion (typically still within the property boundary). In that case, the rate of plume expansion should be estimated (using the methods outlined in the Framework), and then it should be established that there are no current or reasonably foreseeable receptors that would be impacted by this predicted plume expansion.
References
- ^ Interstate Technology and Regulatory Council (ITRC) PFAS Team, 2023. Technical/Regulatory Guidance: Per- and Polyfluoroalkyl Substances. ITRC PFAS Home Page Report pdf
- ^ 2.0 2.1 2.2 2.3 2.4 2.5 Newell, C.J., Adamson, D.T., Kulkarni, P.R., Nzeribe, B.N., Connor, J.A., Popovic, J., and Stroo, H.F., 2021a. Monitored Natural Attenuation to Manage PFAS Impacts to Groundwater: Scientific Basis. Groundwater Monitoring and Remediation, 41(4), pp.76–89. doi: 10.1111/gwmr.12486 Article pdf
- ^ 3.0 3.1 3.2 3.3 Newell, C.J., Adamson, D.T., Kulkarni, P.R., Nzeribe, B.N., Connor, J.A., Popovic, J., and Stroo, H.F., 2021b. Monitored Natural Attenuation to Manage PFAS Impacts to Groundwater: Potential Guidelines. Remediation Journal, 31(4), pp. 7–17. doi: 10.1002/rem.21697 Article pdf
- ^ 4.0 4.1 Adamson, D.T., Kulkarni, P.R., Nickerson, A., Higgins, C.P., Field, J., Schwichtenberg, T., Newell, C., and Kornuc, J.J., 2022. Characterization of relevant site-specific PFAS fate and transport processes at multiple AFFF sites. Environmental Advances, 7, p. 100167. doi: 10.1016/j.envadv.2022.100167 Article pdf
- ^ Brusseau, M.L., 2018. Assessing the Potential Contributions of Additional Retention Processes to PFAS Retardation in the Subsurface. Science of The Total Environment, 613–614, pp. 176–185. doi:10.1016/j.scitotenv.2017.09.065. Article pdf
- ^ Guelfo, J.L., Korzeniowski, S., Mills, M.A., Anderson, J., Anderson, R.H., Arblaster, J.A., Conder, J.M., Cousins, I.T., Dasu, K., Henry, B.J., Lee, L.S., Liu, J., McKenzie, E.R., and Willey, J., 2021. Environmental Sources, Chemistry, Fate, and Transport of Per- and Polyfluoroalkyl Substances: State of the Science, Key Knowledge Gaps, and Recommendations Presented at the August 2019 SETAC Focus Topic Meeting. Environmental Toxicology and Chemistry, 40(12), pp. 3234-3260. doi: 10.1002/etc.5182 Article pdf
- ^ Guo, B., Zeng, J., and Brusseau, M.L., 2020. A Mathematical Model for the Release, Transport, and Retention of Per‐ and Polyfluoroalkyl Substances (PFAS) in the Vadose Zone. Water Resources Research, 56(2), e2019WR026667. doi:10.1029/2019WR026667 Article pdf
- ^ 8.0 8.1 8.2 8.3 8.4 8.5 8.6 Newell, C.J., Javed, H., Li, Y., Johnson, N.W., Richardson, S.D., Connor, J.A., and Adamson, D.T., 2022. Enhanced Attenuation (EA) to Manage PFAS Plumes in Groundwater. Remediation Journal 32(4), pp. 239–257. doi: 10.1002/rem.21731Article pdf
- ^ 9.0 9.1 Newell, C.J., Adamson, D.T., Kulkarni, P.R., Nzeribe, B.N., and Stroo, H., 2020. Comparing PFAS to other groundwater contaminants: Implications for remediation. Remediation Journal, 30(3), pp. 7-26. doi: 10.1002/rem.21645 Article pdf
- ^ Environmental Business Journal (EBJ), 2022. Markets and Technology in Remediation and PFAS, 35 (7/8).
- ^ Salvatore, D., Mok, K., Garrett, K.K., Poudrier, G., Brown, P., Birnbaum, L.S., Goldenman, G., Miller, M.F., Patton, S., Poehlein, M., Varshavsky, J., and Cordner, A., 2022. Presumptive Contamination: A New Approach to PFAS Contamination Based on Likely Sources. Environmental Science and Technology Letters, 9(11), pp.983-990. doi: 10.1021/acs.estlett.2c00502Article pdf
- ^ U.S. Environmental Protection Agency, 2007. Monitored natural attenuation of inorganic contaminants in groundwater, Volume 2 Assessment for Non-Radionuclides Including Arsenic, Cadmium, Chromium, Copper, Lead, Nickel, Nitrate, Perchlorate, and Selenium, Edited by R.G. Ford, R.T. Wilkin, and R.W. Puls. EPA/600/R-07/140. Report pdf
- ^ Chapman, S.W., and Parker, B.L., 2005. Plume persistence due to aquitard back diffusion following dense nonaqueous phase liquid source removal or isolation. Water Resources Research, 41(12), p. W12411. doi: 10.1029/2005WR004224 Article pdf
- ^ Kulkarni, P.R., Adamson, D.T., Popovic, J., and Newell, C.J., 2022. Modeling a Well-Characterized Perfluorooctane Sulfonate (PFOS) Source and Plume Using the REMChlor-MD Model to Account for Matrix Diffusion. Journal of Contaminant Hydrology, 247, p. 103986. doi:10.1016/j.jconhyd.2022.103986Article pdf
- ^ Choi, Y.J., Helbling, D.E., Liu, J., Olivares, C.I., and Higgins, C.P., 2022. Microbial biotransformation of aqueous film-forming foam derived polyfluoroalkyl substances. Science of the Total Environment, 824, p. 153711. doi: 10.1016/j.scitotenv.2022.153711 Article pdf
- ^ Payne, F.C., Quinnan, J.A. and Potter, S.T., 2008. Remediation hydraulics. CRC Press. ISBN-13: 9780849372490/ ISBN-10: 0849372496
- ^ Costanza, J., Arshadi, M., Abriola, L.M., and Pennell, K.D., 2019. Accumulation of PFOA and PFOS at the Air–Water Interface. Environmental Science and Technology Letters, 6(8), pp. 487–91. doi: 10.1021/acs.estlett.9b00355
- ^ Sima, M.W., and Jaffé, P.R., 2021. A Critical Review of Modeling Poly- and Perfluoroalkyl Substances (PFAS) in the Soil-Water Environment. Science of The Total Environment, 757, p. 143793. doi: 10.1016/j.scitotenv.2020.143793
- ^ Zeng, J., and Guo, B., 2023. Reduced Accessible Air–Water Interfacial Area Accelerates PFAS Leaching in Heterogeneous Vadose Zones. Geophysical Research Letters, 50(8), e2022GL102655. doi: 10.1029/2022GL102655Article pdf
- ^ Schaefer, C.E., Nguyen, D., Christie, E., Shea, S., Higgins, C.P., and Field, J.A., 2021. Desorption of poly-and perfluoroalkyl substances from soil historically impacted with aqueous film-forming foam. Journal of Environmental Engineering, 147(2), p. 06020006. doi: 10.1061/(ASCE)EE.1943-7870.0001846
- ^ United States Environmental Protection Agency (USEPA), 1999. Use of Monitored Natural Attenuation at Superfund, RCRA Corrective Action, and Underground Storage Tank Sites. Office of Solid Waste and Emergency Response (OSWER) Directive 9200.4-17P. Report pdf
- ^ Anderson, R.H., Feild, J.B., Dieffenbach-Carle, H., Elsharnouby, O. and Krebs, and R.K., 2022. Assessment of PFAS in collocated soil and porewater samples at an AFFF-impacted source zone: Field-scale validation of suction lysimeters. Chemosphere, 308, p.136247. doi: 10.1016/j.chemosphere.2022.136247
- ^ Brusseau, M.L., and Guo, B., 2023. Revising the EPA dilution-attenuation soil screening model for PFAS. Journal of Hazardous Materials Letters, 4, p. 100077. doi: 10.1016/j.hazl.2023.1000777Article pdf
- ^ Navarro, D.A., Kabiri, S.S., Bowles, K., Knight, E.R., Braeunig, J., Srivastava, P., Boxall, N.J., Douglas, G., Mueller, J., McLaughlin, M.J., and Williams, M., 2024. Review on Methods for Assessing and Predicting Leaching of PFAS from Solid Matrices. Current Pollution Reports, 10(4), pp. 628-647. doi: 10.1007/s40726-024-00326-6 Article pdf
- ^ Adamson, D.T., Nickerson, A., Kulkarni, P.R., Higgins, C.P., Popovic, J., Field, J., Rodowa, A., Newell, C., Deblanc, P., and Kornuc, J.J., 2020. Mass-Based, Field-Scale Demonstration of PFAS Retention within AFFF-Associated Source Areas. Environmental Science and Technology, 54(24), pp. 15768–15777. doi: 10.1021/acs.est.0c04472
- ^ Farhat, S.K., Adamson, D.T., Gavaskar, A.R., Lee, S.A., Falta, R.W., and Newell, C.J., 2020. Vertical Discretization Impact in Numerical Modeling of Matrix Diffusion in Contaminated Groundwater. Groundwater Monitoring and Remediation, 40(2), pp. 52–64. doi: 10.1111/gwmr.12373
- ^ Abrams, S., McGregor, R., Burns, M., Galasso, J., Havranek, T., Hesemann, J., Longsworth, J., McDonough, J., and Mora, R., 2022. PFAS Experts Symposium 2: Statements on available in situ remediation technologies. Remediation Journal, 32(1-2), pp. 45-53. doi: 10.1002/rem.21714
- ^ Divine, C., Heintz, M., Dunn, S., and Pennington, A., 2023. Medical Analogue Framework for Natural Remedies and Passive Risk‐Mitigation Measures in Environmental Remediation. Groundwater Monitoring and Remediation, 43(2), pp. 13-25. doi: 10.1111/gwmr.12579
- ^ Einarson, M.D., and Mackay, D.M., 2001 . Predicting impacts of ground water contamination. Environmental Science and Technology, 35(3), pp. 66A – 73A. doi: 10.1021/es0122647Article pdf