Difference between revisions of "Transition of Aqueous Film Forming Foam (AFFF) Fire Suppression Infrastructure Impacted by Per and Polyfluoroalkyl Substances (PFAS)"
(Tag: Visual edit) |
Debra Tabron (talk | contribs) (Tag: Visual edit) |
||
Line 11: | Line 11: | ||
− | '''Contributor(s):''' Dr. Johnsie Ray Lang, Dr. Jonathan Miles, John Anderson, Dr. Theresa Guillette, [[Craig E. Divine, Ph.D., PG|Dr. Craig Divine]] and [[Dr. Stephen Richardson]] | + | '''Contributor(s):''' [[Dr. Johnsie Ray Lang]], Dr. Jonathan Miles, John Anderson, Dr. Theresa Guillette, [[Craig E. Divine, Ph.D., PG|Dr. Craig Divine]] and [[Dr. Stephen Richardson]] |
Revision as of 20:15, 16 October 2024
Per and polyfluoroalkyl substances (PFAS) contained in Class B aqueous film-forming foams (AFFFs) are known to accumulate on wetted surfaces of many fire suppression systems after decades of exposure[1]. When replacement PFAS-free firefighting formulations are added to existing infrastructure, PFAS can rebound from the wetted surfaces into the new formulations at high concentrations[2][3]. Effective methods are needed to properly transition to PFAS-free firefighting formulations in existing fire suppression infrastructure. Activities to consider when determining the scope of the transition process include (but are not limited to) locating, identifying, and evaluating existing systems and AFFF, fire engineering evaluations, system prioritization, cost/downtime analyses, sampling and analysis, evaluation of risks and hazards to human health and the environment, transportation, and disposal.
Contents
- 1 Introduction
- 2 PFAS Assembly on Solid Surfaces
- 3 Thermodynamics of PFAS Accumulations on Solid Surfaces
- 4 Regulatory Drivers for Transition to PFAS-Free Firefighting Formulations
- 5 Selection of Replacement PFAS-Free Firefighting Formulations
- 6 Selection of Flushing Agent and Approach
- 7 Evaluating PFAS Residuals in the System After Cleaning
- 8 Flushing Media Treatment
- 9 References
- 10 See Also
Related Article(s):
- Perfluoroalkyl and Polyfluoroalkyl Substances (PFAS)
- PFAS Sources
- PFAS Ex Situ Water Treatment
- Supercritical Water Oxidation (SCWO)
- PFAS Treatment by Electrical Discharge Plasma
Contributor(s): Dr. Johnsie Ray Lang, Dr. Jonathan Miles, John Anderson, Dr. Theresa Guillette, Dr. Craig Divine and Dr. Stephen Richardson
Key Resource(s):
- Department of Defense (DoD) performance standard for PFAS-free firefighting formulation: Military Specification MIL-PRF-32725[4]
- Characterization of per- and polyfluoroalkyl substances on fire suppression system piping and optimization of removal methods[1]
Introduction
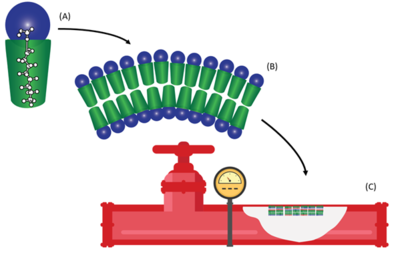
PFAS are a class of synthetic fluorinated compounds which are highly mobile and persistent within the environment[5]. Due to the surfactant properties of PFAS, these compounds self-assemble at any solid-liquid interface forming resilient bilayers during prolonged exposure[6]. Solid phase accumulation of PFAS has been proposed to be influenced by both hydrophobic and electrostatic interactions with fluorinated carbon chain length as the dominant feature influencing sorption[7]. While the majority of previous research into solid phase sorption typically focused on water treatment applications or subsurface porous media[8], recently PFAS accumulations have been identified on the wetted surfaces of fire suppression infrastructure exposed to aqueous film forming foam (AFFF)[1] (see Figure 1).
Fire suppression systems with potential PFAS impacts include fire fighting vehicles that carried AFFF and fixed suppression systems in buildings containing large amounts of flammable materials such as aircraft hangars (Figure 2). PFAS residue on the wetted surfaces of existing infrastructure can rebound into replacement PFAS-free firefighting formulations if not removed during the transition process[2]. Simple surface rinsing with water and low-pressure washing has been proven to be inefficient for removal of surface bound PFAS from piping and tanks that contained fluorinated AFFF[2].
In addition to proper methods for system cleaning to remove residual PFAS, transition to PFAS-free foam may also include consideration of compliance with state and federal regulations, selection of the replacement PFAS-free firefighting formulation, a cost benefit analysis for replacement of the system components versus cleaning, and clean out verification testing. Foam transition should be completed in a manner which minimizes the volume of waste generated as well as preventing any PFAS release into the environment.
PFAS Assembly on Solid Surfaces
The self-assembly of amphiphilic molecules into supramolecular bilayers is a result of their structure and how it interacts with the bulk water of a solution. Single chain hydrocarbon based amphiphiles can form micelles under relatively dilute aqueous concentrations, however for hydrocarbon based surfactants the formation of more complex organized system such as vesicles is rarely seen, requiring double chain amphiphiles such as phospholipids. Associations of single chain cationic and anionic hydrocarbon based amphiphiles into stable supramolecular structures such as vesicles has however been demonstrated[9], with the ion pairing of the polar head groups mimicking the a double tail situation. The behavior of single chain fluorosurfactant amphiphiles has been demonstrated to be significantly different from similar hydrocarbon based analogues. Not only are critical micelle concentrations (CMC) of fluorosurfactants typically two orders of magnitude lower than corresponding hydrocarbon surfactants, but self-assembly can occur even when fluorosurfactants are dispersed at low concentrations significantly below the CMC in water and other solvents[10]. The assembly of fluorinated amphiphiles structurally similar to those found in AFFF have been shown to readily form stable, complex structures including vesicles, fibers, and globules at concentrations as low as 0.5% w/v in contrast to their hydrocarbon analogues which remained fluid at 30% w/v[11][12].
Krafft found that fluorinated amphiphiles formed bilayer membranes with phospholipids, and that the resulting vesicles were more stable than those made of phospholipids alone[13]. The similarities in amphiphilic properties between phospholipids and the hydrocarbon-based surfactants in AFFF suggests that bilayer vesicles may form between these and the fluorosurfactants also present in the concentrate. Krafft demonstrated that both the permeability of resulting mixed vesicles and their propensity to fuse with each other at increasing ionic strength was reduced as a result of the creation of an inert hydrophobic and lipophobic film within the membrane, and also suggested that the fluorinated amphiphiles increased van der Waals interactions in the hydrocarbon region[13]. Thus this low permeability may allow vesicles formed by the surfactants present in AFFF to act as long term repositories of PFAS not only as part of the bilayer itself but also solvated within the vesicle. This prediction is supported by the observation that supramolecular structures formed from single chain fluorinated amphiphiles have been demonstrated to be stable at elevated temperature (15 min at 121°C) and have been shown to be stable over periods of months, even increasing in size over time when stored at environmentally relevant temperatures[12].
Formation of complex structures at relatively low solute concentrations requires the monomer molecules to be well ordered to maintain tight packing in the supramolecular structure[14]. This order results from electrostatic forces, hydrogen bonding, and in the case of fluorinated amphiphiles, hydrophobic interactions. The geometry of the amphiphile also potentially contributes to the type of supramolecular aggregation[15]. Surfactants which adopt a conical shape (such as a typical hydrocarbon based surfactant with a large polar head group and a single alkyl chain as a tail) tend to form micelles more easily. Increasing the bulk of the tail makes the surfactant more cylindrically shaped which makes assembly into bilayers more likely.
Perfluoroalkyl chains are significantly more bulky than their hydrocarbon based analogues both in cross sectional area (28-30 Å2 versus 20 Å2, respectively) and mean volume (CF2 and CF3 estimated as 38 Å3 and 92 Å3 compared to 27 Å3 and 54 Å3 for CH2 and CH3)[13][10]. Structural studies on linear PFOS have shown that the molecule adopts an unusual helical structure[16][17] in aqueous and solvent phases to alleviate steric hindrance. This arrangement results from the carbon chain starting in the planar all anti conformation and then successively twisting all the CC-CC dihedrals by 15°-20° in the same direction[18]. The conformation also minimizes the electrostatic repulsion between fluorine atoms bonded to the same side of the carbon backbone by maximizing the interatomic distances between them[17].
A consequence of the helical structure is that there is limited carbon-carbon bond rotation within the perfluoroalkyl chain giving them increased rigidity compared to alkyl chains[19]. The bulkiness of the perfluoroalkyl chain confers a cylindrical shape on the fluorosurfactant amphiphile and therefore favors the formation of bilayers and vesicles the aggregation of which is further assisted by the rigidity of the molecules which allow close packing in the supramolecular structure. Fluorosurfactants therefore cannot be regarded as more hydrophobic analogues of hydrogenated surfactants. Their self-assembly behavior is characterized by a strong tendency to form vesicles and lamellar phases rather than micelles, due to the bulkiness and rigidity of the perfluoroalkyl chain that tends to decrease the curvature of the aggregates they form in solution[20]. The larger tail cross section of fluorinated compared to hydrogenated amphiphiles tends to favor the formation of aggregates with lesser surface curvature, therefore rather than micelles they form bilayer membranes, vesicles, tubules and fibers[11][21][22][23]. Rojas et al. (2002) demonstrated that perfluorooctyl sulphonamide formed a contiguous bilayer at 50 mg/L with self-assembled aggregates present at concentrations as low as 10 mg/L[24].
Thermodynamics of PFAS Accumulations on Solid Surfaces
The thermodynamics of formation of amphiphiles into supramolecular species requires consideration of both hydrophobic and hydrophilic interactions resulting from the amphoteric nature of the molecule. The hydrophilic portions of the molecule are driven to maximize their solvation interaction with as many water molecules as possible, whereas the hydrophobic portions of the molecule are driven to aggregate together thus minimizing interaction with the bulk water. Both of these processes change the enthalpy and entropy of the system.
In aqueous solution, the hydrophilic portions of an amphiphile form hydrogen bonds (4 - 120 kJ/mol) and van der Waals interactions (<5 kJ/mol) with water molecules and surfaces, and electrostatic interactions (5 – 300 kJ/mol) can also occur where the amphiphile is ionic[25]. These interactions, although weak compared to intramolecular covalent bonds within a molecule are energetically favorable and increase the enthalpy of the combined solute-solvent system. Thus, the hydrophilic portion of an amphiphile will look to maximize enthalpic gain through hydrogen bond interactions with the bulk water.
The hydrophobic portion of an amphiphile cannot form hydrogen bonds with the bulk solution, and its presence disrupts the hydrogen bond interactions between individual water molecules within the bulk water matrix. This disruption lowers the entropy of the system by reducing the degrees of translational rotational freedom available to the bulk water. The second law of thermodynamics dictates that a system will arrange itself to maximize its entropy. With hydrophobic species this can be achieved by their spontaneous aggregation, as the reduction in solution entropy of the aggregated system is less than that which would occur if the component parts were solvated individually. These hydrophobic and hydrophilic interactions are weak, and the individual entropy gain per amphiphile upon aggregation is very small. However, taken together the overall effect on the entropy of the aggregate is sufficient to maintain it in solution, and moreover these interactions make the aggregates resistant to minor perturbations while retaining the reversibility of the self-assembled structure[25].
Regulatory Drivers for Transition to PFAS-Free Firefighting Formulations
Regulations restricting the use and release of PFAS are being proposed and promulgated worldwide, with several enacted regulations addressing the use of aqueous film forming foams (AFFF) containing PFAS[26][27][28][29][30][31][32][33]. In addition to regulated usage, firefighting formulation users are transitioning to PFAS-free firefighting formulations to reduce environmental liability in the event of a release, to reduce the cost of expensive containment systems and management of generated waste streams, and to avoid reputational damage. In 2016, Queensland, Australia was one of the first governments to ban PFAS use in firefighting foam[26]. The US 2020 National Defense Authorization Act specified immediate prohibition of controlled releases of AFFF containing PFAS and required the Secretary of the Navy to publish a specification for PFAS-free firefighting formulation use and ensure it is available for use by the Department of Defense (DoD) by October 1, 2023[34]. The National Fire Protection Association (NFPA) recently removed the requirement for AFFF containing PFAS from their Standard on Aircraft Hangars and added two new chapters to allow users to determine if AFFF containing PFAS is needed at their facility[35].
Selection of Replacement PFAS-Free Firefighting Formulations
Since they first entered the market in the 2000s, the operational capabilities of PFAS-free firefighting formulations have grown[36] and numerous companies are now manufacturing and delivering PFAS-free firefighting formulations for fixed systems and AFFF vehicles[37][38][39]. Key factors in the selection of a PFAS-free firefighting formulation product are compatibility of the new formulation with the existing system (as confirmed by a fire protection engineer) and environmental certifications (i.e., verifying the absence of organic fluorine or PFAS or the absence of other non-fluorine environmental contaminants).
In January 2023, the US Department of Defense (USDoD) published the Performance Specification for Fire Extinguishing Agent, Fluorine-Free Foam (F3) Liquid Concentrate for Land-Based, Fresh Water Applications[4]. This Military Performance Specification (Mil-Spec) allows PFAS-free firefighting formulations to be certified as meeting certain standardized operational goals for use in military settings. There are now several certified PFAS-free firefighting formulations listed on the USDoD’s Qualified Products List (QPL). In addition to Mil-Spec requirements, PFAS-free firefighting formulations can also be certified through Underwriters Laboratories Standard for Safety, Foam Equipment and Liquid Concentrates, UL 162, which requires the new firefighting formulations be investigated for suitability and compatibility with the specific equipment with which they are intended to be used[40]. Several PFAS-free foams have been certified under various parts of EN1568, the European Standard which specifies the necessary foam properties and performance requirements[41]. Both ESTCP and SERDP have supported (and continue to support) the development and field validation of PFAS-free firefighting formulations (e.g. WP22-7456, WP21-3465, WP20-1535). Both the US Federal Aviation Administration (FAA) and National Fire Protection Association (NFPA) have performed a variety of foam certification tests on numerous PFAS-free firefighting formulations[42][43].
Selection of Flushing Agent and Approach
General industry guidance has typically recommended several flushes with water to remove PFAS from impacted equipment. Owing to the unique physical and chemical properties of PFAS, the use of room temperature water as the primary flushing agent to remove PFAS from impacted equipment has been demonstrated to not be effective enough. To address these recalcitrant accumulations, companies are developing new methods to remove self-assembled PFAS bilayers from existing fire-fighting infrastructure so that it can be successfully transitioned to PFAS-free formulations. Arcadis developed a non-toxic cleaning agent, Fluoro FighterTM, which has been demonstrated to be effective for removal of PFAS from equipment by disrupting the accumulated layers of PFAS coating the AFFF-wetted surfaces.
Laboratory studies have supported the optimization of this PFAS removal method in fire suppression system piping obtained from a commercial airport hangar in Sydney, Australia[1]. Prior to removal from the hangar, the stainless-steel pipe held PFAS-containing AFFF for more than three decades. Results indicated that Fluoro FighterTM, as well as flushing at elevated temperatures, removed more surface associated PFAS in comparison to equivalent extractions using methanol or water at room temperature. ESTCP has supported (and continues to support) the development and field validation of best practices for methodologies to clean foam delivery systems (e.g. ER20-5364, ER20-5361, ER20-5369, ER21-7229).
Evaluating PFAS Residuals in the System After Cleaning
In general, PFAS sampling techniques used to support firefighting formulation transition activities are consistent with conventional sampling techniques used in the environmental industry, but special consideration is made regarding high concentration PFAS materials, elevated detection levels, cross-contamination potential, precursor content, and matrix interferences. The analytical method selected should be appropriate for the regulatory requirements in the site area.
Arcadis implemented its proprietary cleaning process on foam concentrate piping and foam concentrate storage tanks at Naval Air Station Willow Grove utilizing Fluoro FighterTM. The system had two identical foam concentrate tanks, but one tank was isolated from the cleaning agent flow path, preserving this tank in an uncleaned state as a negative control for the experiment. The total PFAS concentrations measured by Total Oxidizable Precursor (TOP) Assay in the PFAS-free firefighting formulation rebound samples were 12 µg/L in the cleaned tank compared to ~1,500 µg/L in the uncleaned tank (Figure 3) indicating this cleaning process results in orders of magnitude reductions in PFAS rebound concentrations.
Flushing Media Treatment
Numerous technologies for treatment of PFAS-impacted water sources, including flushing agents, have been and are currently being developed. These include separation technologies such as foam fractionation, nanofiltration, sorbents/flocculants, ion exchange resins, reverse osmosis, and destructive technologies such as sonolysis, electrochemical oxidation, hydrothermal alkaline treatment, enhanced contact plasma, and supercritical water oxidation (SCWO). Many of these technologies have rapidly developed from bench-scale (e.g., microcosms, columns, single reactors) to commercially available field-scale units capable of managing PFAS-impacted waters of varying waste volumes and PFAS compositions and concentrations. Ongoing field research continues to improve the treatment efficiency, reliability, and versatility of these technologies, both individually and as coupled treatment solutions (e.g., treatment train). ESTCP has supported (and continues to support) the development and field validation of separation and destructive technologies for treatment of PFAS-impacted water sources, including flushing agents (e.g. ER20-5370, ER20-5369, ER20-5350, ER20-5355).
Remedy selection for treatment of flushing agents involves several key factors. It is critical that environmental practitioners have up-to-date technical and practical knowledge on the suitability of these remedial options for different site conditions, treatment volumes, PFAS composition (e.g., presence of precursors, co-contaminants), PFAS concentrations, safety considerations, potential for undesired byproducts (e.g., perchlorate, disinfection byproducts), and treatment costs (e.g., energy demand, capital costs, operational labor).
References
- ^ 1.0 1.1 1.2 1.3 Lang, J.R., McDonough, J., Guillette, T.C., Storch, P., Anderson, J., Liles, D., Prigge, R., Miles, J.A.L., Divine, C., 2022. Characterization of per- and polyfluoroalkyl substances on fire suppression system piping and optimization of removal methods. Chemosphere, 308(Part 2), 136254. doi: 10.1016/j.chemosphere.2022.136254 Article pdf
- ^ 2.0 2.1 2.2 Ross, I., and Storch, P., 2020. Foam Transition: Is It as Simple as "Foam Out / Foam In?". The Catalyst (Journal of JOIFF, The International Organization for Industrial Emergency Services Management), Q2 Supplement, 20 pages. Industry Newsletter
- ^ Kappetijn, K., 2023. Replacement of fluorinated extinguishing foam: When is clean clean enough? The Catalyst (Journal of JOIFF, The International Organization for Industrial Emergency Services Management), Q1 2023, pp. 31-33. Industry Newsletter
- ^ 4.0 4.1 US Department of Defense, 2023. Performance Specification for Fire Extinguishing Agent, Fluorine-Free Foam (F3) Liquid Concentrate for Land-Based, Fresh Water Applications. Mil-Spec MIL-PRF-32725, 18 pages. Military Specification Document
- ^ Giesy, J.P., Kannan, K., 2001. Global Distribution of Perfluorooctane Sulfonate in Wildlife. Environmental Science and Technology 35(7), pp. 1339-1342. doi: 10.1021/es001834k
- ^ Krafft, M.P., Riess, J.G., 2015. Selected physicochemical aspects of poly- and perfluoroalkylated substances relevant to performance, environment and sustainability-Part one. Chemosphere, 129, pp. 4-19. doi: 10.1016/j.chemosphere.2014.08.039
- ^ Higgins, C.P., Luthy, R.G., 2006. Sorption of Perfluorinated Surfactants on Sediments. Environmental Science and Technology, 40(23), pp. 7251-7256. doi: 10.1021/es061000n
- ^ Brusseau, M.L., 2018. Assessing the Potential Contributions of Additional Retention Processes to PFAS Retardation in the Subsurface. Science of the Total Environment, 613-614, pp. 176-185. doi: 10.1016/j.scitotenv.2017.09.065 Article pdf
- ^ Fukuda, H., Kawata, K., Okuda, H., 1990. Bilayer-Forming Ion-Pair Amphiphiles from Single-Chain Surfactants. Journal of the American Chemical Society, 112(4), pp. 1635-1637. doi: 10.1021/ja00160a057
- ^ 10.0 10.1 Krafft, M.P., 2006. Highly fluorinated compounds induce phase separation in, and nanostructuration of liquid media. Possible impact on, and use in chemical reactivity control. Journal of Polymer Science Part A: Polymer Chemistry, 44(14), pp. 4251-4258. doi: 10.1002/pola.21508 Article pdf
- ^ 11.0 11.1 Krafft, M.P., Guilieri, F., Riess, J.G., 1993. Can Single-Chain Perfluoroalkylated Amphiphiles Alone form Vesicles and Other Organized Supramolecular Systems? Angewandte Chemie International Edition in English, 32(5), pp. 741-743. doi: 10.1002/anie.199307411
- ^ 12.0 12.1 Krafft, M.P., Guilieri, F., Riess, J.G., 1994. Supramolecular assemblies from single chain perfluoroalkylated phosphorylated amphiphiles. Colloids and Surfaces A: Physicochemical and Engineering Aspects, 84(1), pp. 113-119. doi: 10.1016/0927-7757(93)02681-4
- ^ 13.0 13.1 13.2 Krafft, M.P., Riess, J.G., 1998. Highly Fluorinated Amphiphiles and Collodial Systems, and their Applications in the Biomedical Field. A Contribution. Biochimie, 80(5-6), pp. 489-514. doi: 10.1016/S0300-9084(00)80016-4
- ^ Ringsdorf, H., Schlarb, B., Venzmer, J., 1988. Molecular Architecture and Function of Polymeric Oriented Systems: Models for the Study of Organization, Surface Recognition, and Dynamics of Biomembranes. Angewandte Chemie International Edition in English, 27(1), pp. 113-158. doi: 10.1002/anie.198801131
- ^ Israelachvili, J.N., Mitchell, D.J., Ninham, B.W., 1976. Theory of Self-Assembly of Hydrocarbon Amphiphiles into Micelles and Bilayers. Journal of the Chemical Society, Faraday Transactions 2: Molecular and Chemical Physics, 72, pp. 1525-1568. doi: 10.1039/F29767201525
- ^ Erkoç, Ş., Erkoç, F., 2001. Structural and electronic properties of PFOS and LiPFOS. Journal of Molecular Structure: THEOCHEM, 549(3), pp. 289-293. doi:10.1016/S0166-1280(01)00553-X
- ^ 17.0 17.1 Torres, F.J., Ochoa-Herrera, V., Blowers, P., Sierra-Alvarez, R., 2009. Ab initio study of the structural, electronic, and thermodynamic properties of linear perfluorooctane sulfonate (PFOS) and its branched isomers. Chemosphere 76(8), pp. 1143-1149. doi: 10.1016/j.chemosphere.2009.04.009
- ^ Abbandonato, G., Catalano, D., Marini, A., 2010. Aggregation of Perfluoroctanoate Salts Studied by 19F NMR and DFT Calculations: Counterion Complexation, Poly(ethylene glycol) Addition, and Conformational Effects. Langmuir, 26(22), pp. 16762-16770. doi: 10.1021/la102578k
- ^ Barton, S.W., Goudot, A., Bouloussa, O., Rondelez, F., Lin, B., Novak, F., Acero, A., Rice, S., 1992. Structural transitions in a monolayer of fluorinated amphiphile molecules. The Journal of Chemical Physics, 96(2), pp. 1343-1351. doi: 10.1063/1.462170
- ^ Barton, C.A., Butler, L.E., Zarzecki, C.J., Flaherty, J., Kaiser, M., 2006. Characterizing Perfluorooctanoate in Ambient Air near the Fence Line of a Manufacturing Facility: Comparing Modeled and Monitored Values. Journal of the Air and Waste Management Association, 56, pp. 48-55. doi: 10.1080/10473289.2006.10464429 Article pdf
- ^ Furuya, H., Moroi, Y., Kaibara, K., 1996. Solid and Solution Properties of Alkylammonium Perfluorocarboxylates. The Journal of Physical Chemistry, 100(43), pp. 17249-17254. doi: 10.1021/jp9612801
- ^ Giulieri, F., Krafft, M.P., 1996. Self-organization of single-chain fluorinated amphiphiles with fluorinated alcohols. Thin Solid Films, 284-285, pp. 195-199. doi: 10.1016/S0040-6090(95)08304-9
- ^ Gladysz, J.A., Curran, D.P., Horvath, I.T., 2004. Handbook of Fluorous Chemistry. WILEY-VCH Verlag GmbH & Co. KGaA,, Weinheim, Germany. ISBN: 3-527-30617-X
- ^ Rojas, O.J., Macakova, L., Blomberg, E., Emmer, A., and Claesson, P.M., 2002. Fluorosurfactant Self-Assembly at Solid/Liquid Interfaces. Langmuir, 18(21), pp. 8085-8095. doi: 10.1021/la025989c
- ^ 25.0 25.1 Lombardo, D., Kiselev, M.A., Magazù, S., Calandra, P., 2015. Amphiphiles Self-Assembly: Basic Concepts and Future Perspectives of Supramolecular Approaches. Advances in Condensed Matter Physics, vol. 2015, article ID 151683, 22 pages. doi: 10.1155/2015/151683 Article pdf
- ^ 26.0 26.1 Queensland (Australia) Department of Environment and Heritage Protection, 2016. Operational Policy - Environmental Management of Firefighting Foam. 16 pages. Policy pdf
- ^ U.S. Congress, 2019. S.1790 - National Defense Authorization Act for Fiscal Year 2020. United States Library of Congress. Text and History of Law.
- ^ Arizona State Legislature, 2019. Title 36, Section 1696. Firefighting foam; prohibited uses; exception; definitions. Text of Law
- ^ California Legislature, 2020. Senate Bill No. 1044, Chapter 308, Firefighting equipment and foam: PFAS chemicals. Text and History of Law
- ^ Arkansas General Assembly, 2021. An Act Concerning the Use of Certain Chemicals in Firefighting Foam; and for Other Purposes. Act 315, State of Arkansas. Text and History of Law.
- ^ Espinosa, Summers, Kelly, J., Statler, Hansen, Young, 2021. Amendment to Fire Prevention and Control Act. House Bill 2722. West Virginia Legislature. Text and History of Law
- ^ Louisiana Legislature, 2021. Act No. 232. Text and History of Law
- ^ Vermont Legislature, 2021b. Act No. 36, PFAS in Class B Firefighting Foam. Text and History of Law
- ^ U.S. Congress, 2021. S.2792 - National Defense Authorization Act for Fiscal Year 2021. United States Library of Congress. Text and History of Law.
- ^ National Fire Protection Association (NFPA), 2022. Codes and Standards, 409: Standard on Aircraft Hangars. NFPA Website
- ^ Allcorn, M., Bluteau, T., Corfield, J., Day, G., Cornelsen, M., Holmes, N.J.C., Klein, R.A., McDowall, J.G., Olsen, K.T., Ramsden, N., Ross, I., Schaefer, T.H., Weber, R., Whitehead, K., 2018. Fluorine-Free Firefighting Foams (3F) – Viable Alternatives to Fluorinated Aqueous Film-Forming Foams (AFFF). White Paper prepared for the IPEN by members of the IPEN F3 Panel and associates, POPRC-14, Rome. Article pdf.
- ^ Ansul (Company), Ansul NFF-331 3%x3% Non-Fluorinated Foam Concentrate (Commercial Product). Product Data Sheet.
- ^ BioEx (Company), Ecopol A+ (Commercial Product). Website
- ^ National Foam (Company), 2020. Avio F3 Green KHC 3%, Fluorine Free Foam Concentrate (Commercial Product). Safety Data Sheet
- ^ Underwriters Laboratories Inc., 2018. UL162, UL Standard for Safety, Foam Equipment and Liquid Concentrates, 8th Edition, Revised 2022. 40 pages. Website
- ^ European Standards, 2018. CSN EN 1568-1 ed. 2: Fire extinguishing media - Foam concentrates - Part 1: Specification for medium expansion foam concentrates for surface application to water-immiscible liquids. 48 pages. European Standards Website.
- ^ Back, G.G., Farley, J.P., 2020. Evaluation of the Fire Protection Effectiveness of Fluorine Free Firefighting Foams. National Fire Protection Association, Fire Protection Research Foundation. Report pdf.
- ^ Casey, J., Trazzi, D., 2022. Fluorine-Free Foam Testing. Federal Aviation Administration (FAA) Final Report. Article pdf
See Also
Connecticut Take-Back Program for municipal fire departments using AFFF containing PFAS
Arcadis blog on Fluoro FighterTM
Project Summary ESTCP ER20-5350: Supercritical Water Oxidation (SCWO) for Complete PFAS Destruction