Difference between revisions of "User:Debra Tabron/sandbox"
Debra Tabron (talk | contribs) |
Debra Tabron (talk | contribs) |
||
Line 14: | Line 14: | ||
'''Key Resource(s)''': | '''Key Resource(s)''': | ||
*[https://doi.org/10.1111/j.1745-6584.2009.00654.x Crude oil at the Bemidji site: 25 years of monitoring, modeling, and understanding]<ref name= "Essaid2011">Essaid, H.I., Bekins, B.A., Herkelrath, W.N. and Delin, G.N., 2011. Crude oil at the Bemidji site: 25 years of monitoring, modeling, and understanding. Groundwater, 49(5), pp.706-726. [https://doi.org/10.1111/j.1745-6584.2009.00654.x doi: 10.1111/j.1745-6584.2009.00654.x]</ref> | *[https://doi.org/10.1111/j.1745-6584.2009.00654.x Crude oil at the Bemidji site: 25 years of monitoring, modeling, and understanding]<ref name= "Essaid2011">Essaid, H.I., Bekins, B.A., Herkelrath, W.N. and Delin, G.N., 2011. Crude oil at the Bemidji site: 25 years of monitoring, modeling, and understanding. Groundwater, 49(5), pp.706-726. [https://doi.org/10.1111/j.1745-6584.2009.00654.x doi: 10.1111/j.1745-6584.2009.00654.x]</ref> | ||
− | *[https://doi.org/ | + | *[https://doi.org/10.1111/gwat.12419 Crude Oil Metabolites in Groundwater at Two Spill Sites]<ref name= "Bekins2016">Bekins, B.A., Cozzarelli, I.M., Erickson, M.L., Steenson, R.A. and Thorn, K.A., 2016. Crude oil metabolites in groundwater at two spill sites. Groundwater, 54(5), pp.681-691. [https://doi.org/10.1111/gwat.12419 doi: 10.1111/gwat.12419]</ref> |
==Introduction== | ==Introduction== |
Revision as of 19:20, 13 June 2019
A long-term study of a 40-year-old crude oil spill provides insights about petroleum hydrocarbon natural attenuation processes and rates. In the source zone, fermentation coupled to methanogenesis is the dominant natural source zone depletion (NSZD) process, and most of the carbon mass exits the surface as CO2 efflux. Monitored natural attenuation (MNA) of the groundwater plume shows that benzene degradation is coupled to iron reduction and that the benzene plume is stable. A plume of hydrocarbon oxidation products measured as nonvolatile dissolved organic carbon (NVDOC) expanded ~20 m in 20 years. Most of the NVDOC is biodegraded by 200 m from the source, but optical data suggest there are components that persists for 300 m. Biological effects screening indicates decreasing biological effects with distance from the source.
Related Article(s):
- Natural Source Zone Depletion (NSZD)
- Monitored Natural Attenuation (MNA)
- Biodegradation - Hydrocarbons
CONTRIBUTOR(S): Dr. Barbara Bekins
Key Resource(s):
- Crude oil at the Bemidji site: 25 years of monitoring, modeling, and understanding[1]
- Crude Oil Metabolites in Groundwater at Two Spill Sites[2]
Introduction
The purpose of this article is to provide an overview of a petroleum release research site and to summarize key results relating to natural source zone depletion (NSZD) and to anaerobic degradation of hydrocarbon plumes. A final section briefly describes ongoing research projects at the site.
The Bemidji crude oil research site was established by the U. S. Geological Survey’s Toxics Substance Hydrology Program in 1983 at the location of an oil release which occurred in 1979 when a high-pressure pipeline ruptured. The goal of the research is to improve understanding of monitored natural attenuation (MNA) of petroleum hydrocarbon spills with transformative research on the source, transport and fates of the major contaminant classes. To date, 274 publications by interdisciplinary teams working at the site have covered physical, biological, and geochemical processes controlling these contaminants[3]. Since 2009, the pipeline owner, Enbridge Energy LLC, has provided supplemental funding for site management and research seed money through a collaborative agreement between USGS, Minnesota Pollution Control Agency, and Beltrami County.
Site Description
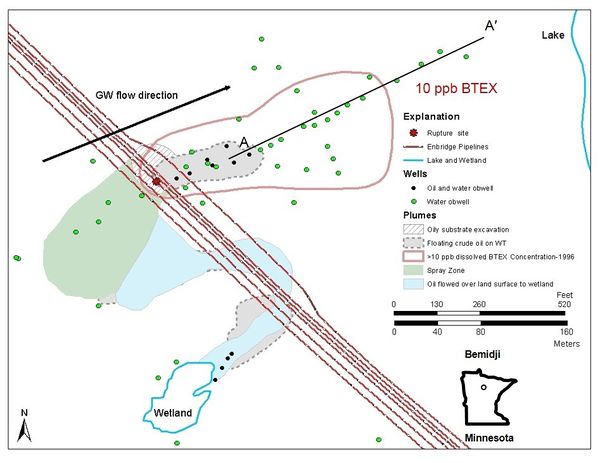
The site (Figure 1) is located 16 km northwest of Bemidji, MN. An oil release occurred on August 20, 1979, when a high-pressure pipeline ruptured along a seam, spilling 10,700 barrels (1.7 million liters) of light, low sulfur crude. The immediate cleanup removed about 70% of the oil by pumping, excavation, land farming, and burning. An area of 6,500 m2 that was sprayed by oil from the pipeline contains oil in the top few centimeters of soil. The remaining oil infiltrated into the aquifer forming three oil bodies at the water table designated as the north, middle and south pools. Additional remediation by a dual-pump recovery system from 1999 through 2003 removed an estimated 36-41% of the oil from the three oil pools[5].
Most studies of the Bemidji source zone and plume have been focused on the north pool. The oil body at the water table is 1-2 m thick and spans an area of 100 m × 25 m. Oil saturations are 10-70%, with the highest saturation in a coarse-grained layer below the water table[1]. Residual oil saturations of 15-30% are present from the land surface to the water table[6].
The depth to the water table near the north pool is 6-8 m. The aquifer is a glacial outwash deposit of medium to coarse variably sorted sand with <0.1% organic carbon. It is underlain by poorly permeable till at 18-27 m depth. The range of estimated hydraulic conductivities is 0.56-7 x 10-5 m/s[1]. The background groundwater is aerobic with dissolved oxygen of 9 mg/L[7]. Average pore water velocity estimates range from 0.004 to 0.056 m/day (0.5-67 ft/year, 0.1-20.5 m/year)[7].
Natural Source Zone Depletion (NSZD)
Natural Source Zone Depletion (NSZD) refers to the changes over time in the composition of spilled oil or fuel that occurs naturally by dissolution, volatilization and biodegradation[10]. Figure 2 shows a conceptual model of NSZD processes at the Bemidji site. Biodegradation within the oil body occurs primarily by fermentation coupled to methanogenesis[11][12][13]. The biogenic methane produced in the oil body diffuses toward the land surface where it is oxidized in the middle of the vadose zone by methanotrophic bacteria[14]. The CO2 produced by methane oxidation together with some produced in the source zone exits as surface efflux over the oil body[15]. Garg et al., 2017[10] present a useful summary of the important NSZD results from the site. This brief section will focus on how NSZD has been measured at the north pool and what has been discovered. Estimates of NSZD at Bemidji have been based on a variety of indicators, including vadose zone gas concentrations, compositions of oil samples from wells, surface CO2 efflux, temperature measurements, and modeling.
Gas concentration data from 1985, six years after the spill, indicated that volatilization accounted for 97% of NSZD and biodegradation only 3%. The volatile fraction was dominated by C3-C5 alkanes and was oxidized in the vadose zone before reaching the surface[16]. Total losses were estimated to be 10.5 kg/day. By 1997, volatilization losses were 10 times lower and although biodegradation losses increased, total losses had dropped to 2 kg/day. The total average mass loss (Table 1) was estimated from the gas data to be 0.6% per year over the period 1979-1997[17].
Analyses of oil samples collected in 2010 from 13 wells in the north oil pool showed that 31 years after the spill, n-alkanes, toluene, and o-xylene were the most depleted hydrocarbons, while n-C10–24 cyclohexanes, tri- and tetra- methylbenzenes, acyclic isoprenoids, and naphthalenes were the least depleted. Benzene was still present at every sampling location. The technique of normalizing composition to a conserved compound yielded estimates for spatially variable mass losses ranging from 18% to 30% or an overall average rate of 0.8% per year (Table 1)[18].
Reference | Method | Time Interval | Equivalent CO2 flux | Total Oil Loss Rate | Annual Loss | Comments | |
---|---|---|---|---|---|---|---|
μmol/m2-s | kg/m2-d | gal/acre-y | %/y | ||||
Chaplin et al. (2002)[17] | Gas data and 1D model | 1979-1997 | 0.75 | 9.33×10-4 | 429 | 0.63 | Published estimate of 0.2% was based on total oil rather than N. Pool; Loss given is average of 3 oil sites in their Table 4. |
Baedecker et al. (2018)[19] | Oil composition | 1979-2010 | 0.93 | 1.16×10-3 | 533 | 0.79 | Average using four conservative compounds. Computed from published values as (7×30.1+6×17.8)/13÷31 years. |
Sihota et al. (2016)[9] | Surface CO2 efflux | 2011-2013 | 1.1 | 1.37×10-3 | 627 | 0.93 | Value given is annual average efflux June 2011-July 2013 from their Table 1. |
Molins et al. (2010)[20] | Gas and water data with 2D model | 1979-2007 | 2.15 | 2.67×10-3 | 1,226 | 1.81 | Value given is total flux. Model lower boundary methane flux of 1.3 mol/m2-d was 0.7 of total. Published value based on only VZ degradation was 1% y-1. |
Ng et al. (2015)[8] | 2D model using oil, gas, and water data | 1979-2008 | 1.46 | 1.81×10-3 | 831 | 1.23 | Oil loss rate computed from losses given in their Figure 7, masses in Table 3, and 82 m length of oil body from Figure 2. |
* Note: The values in bold are taken directly from the listed reference. The other columns are computed from this column using the following values: oil specific gravity = 0.85 [21], carbon fraction of oil = 0.835[19], north pool oil body area = 2,323 m2 and total mass of oil 124,950 kg[22]. |
The CO2 efflux technique involves measuring the flux of CO2 leaving the surface above the source zone and comparing it to an average rate from natural soil respiration in uncontaminated locations. The difference in efflux between the source zone and background is assumed to reflect the amount generated by biodegradation of the source, but the estimate can be complicated by spatial variation in background rates[15]. A refinement of the technique is based on the principle that degradation of oil produces ancient carbon, and analyzing for 14C content of CO2 provides more precise estimates of the efflux attributable to contaminant degradation[23]. Monthly data from the site shows that surface efflux and subsurface gas concentrations vary seasonally, with 2-3 times higher efflux values in the summer and fall compared to the spring and winter[9]. The CO2 effluxes are typically expressed in units of micromoles of CO2 per meter-squared per second (µmol m 2 s 1). The average annual rate measured for the north pool from June 2011 until July 2013 was 1.1 ± 0.4 µmol m 2 s 1. This corresponds to an average oil biodegradation rate of 0.9% per year (Table 1).
The temperature method for evaluating NSZD involves measuring vertical profiles of vadose zone temperatures above the source zone and at a background site subject to the same external heating. The source of the heat at the Bemidji site is the combined effect of methane oxidation in the vadose zone above the oil body and the active oil pipelines passing through the site. Temperature increases are used to estimate NSZD rates by converting the excess heat to reaction rates using the reaction enthalpy. Estimates using average annual temperature increases were comparable to NSZD rates estimated from the average annual surface efflux of CO2.. However, modeling indicates that half of the excess heat measured in the aquifer came from the pipelines, underscoring the importance of subtracting temperature effects of nearby infrastructure before using excess temperatures to estimate NSZD rates[24]. The method has been carefully tested at a refined petroleum products terminal, where researchers found 8% higher estimated NSZD rates using CO2 flux measurements compared to temperature based estimates.
Two comprehensive modeling studies have focused on NSZD at the site[20]. Molins et al., 2010[20] modeled the NSZD processes of volatilization, biodegradation and dissolution in the vadose zone. They used gas and aqueous concentration data with estimates of vadose zone transport properties, but did not have surface efflux or oil composition data to constrain fluxes. Their calibrated methane efflux was 2.15 µmol m 2 s 1, of which 30% came from inside the model and 70% from the smear zone. Within the model domain the modeled loss was 1% per year during the period 1979 – 2007. Including the flux and oil outside the model domain gives a loss of 1.8% per year (Table 1). An important conclusion of this paper is that soil diffusion properties are poorly constrained, and surface efflux measurements might be an effective strategy to constrain gas flux. The model by Ng et al., 2015[8] included biodegradation and dissolution constrained by surface efflux and oil composition data but not volatilization. Their overall loss was 1.8x10-3 kg/m2-d during 1979 – 2008 or 1.2% per year (Table 1). A major finding of this study is that 85% of the carbon loss from the oil occurs through CO2 gas efflux from the surface over the source zone[25].
NSZD rates estimated with CO2 efflux and temperature increases are sometimes used to estimate the volume of oil or mass of total petroleum hydrocarbons (TPH) degraded per year. Conversion to volume of LNAPL requires knowing the mass fraction of C and specific gravity of the LNAPL, while conversion to mass of TPH is sometimes based on a model hydrocarbon such as decane (C10H22)[15]. However, the CO2 efflux and heat are generated by the biodegradation of a subset of the compounds in the oil. Baedecker et al., 2011[18][19] showed that some compounds degrade much faster than others. Figure 3 shows model estimates for the amount of degradation by 2008 for each major class of oil compounds[8]. Biodegradation of the n-alkane fraction contributes most of the CO2 efflux[8] and associated heat, which may have limited relevance for risk evaluations.
The differences between the loss rates of various compound classes are due to several factors. For example, the n-alkanes degrade in place under methanogenic conditions[26] without migrating in the aqueous phase[8]. Losses are fastest below a local topographic depression where focused recharge transports nitrate to the oil body[11][18][19]. However, the alkylbenzenes dissolve in the groundwater before degrading. Thus, their loss rates vary with effective solubility and susceptibility to methanogenic degradation. For example, benzene and ethylbenzene do not degrade under methanogenic conditions at this site, and their losses are controlled by solubility and oil pore-space saturation[27]. Although benzene degradation under methanogenic conditions has been demonstrated in the lab[28], a literature review concluded it is unreliable in the field[29]. The higher effective solubility of benzene has resulted in greater cumulative losses than for ethylbenzene[18]. For alkylbenzenes that degrade under methanogenic conditions, losses are accelerated by methanogenic degradation in the aqueous phase adjacent to the oil, which lowers concentrations driving further dissolution[30].
Natural Attenuation of the Groundwater Plume
A groundwater contaminant plume extends 335 m downgradient from the north oil pool to the shore of a small unnamed lake[31][32]. Essaid et al., 2011[1] present a comprehensive summary of processes in the plume. This brief review will focus on the key biodegradation processes, the importance of anaerobic biodegradation, and recent work on partial transformation products of hydrocarbons found in the plume.
Figure 4 shows a simplified schematic of redox zones for a cross section of the north pool plume from Cozzarelli, et al., 2016[33]. Although the background groundwater is aerobic with 8-10 mg/L dissolved oxygen, the plume core is anaerobic. The anaerobic conditions are maintained by the ongoing supply of 40-50 mg/L total dissolved organic carbon entering the groundwater from the oil[34], together with limited mixing with aerobic water on the plume fringes[35]. The dominant redox processes in the plume core are iron reduction and fermentation coupled to methanogenesis; with nitrate and sulfate reduction being relatively unimportant[36]. During iron reduction, the Fe(III) in poorly crystalline iron oxyhydroxide coatings on the aquifer sediments is reduced to Fe(II)[37] of which 99% is locally redeposited onto the aquifer sediments[25]. Over the last 40 years the sediment Fe(III) coatings have been almost completely consumed near the source zone and in the center of the plume[38]. Accordingly, the methanogenic zone has expanded and the iron-reducing zone has moved approximately 60 m downgradient in the 10-year interval 1996-2006[8][38][39]. Aerobic degradation occurs on the plume fringes[40], but a high resolution push-probe survey shows it can account for at most 15% of the reduced carbon oxidation[38]. A modeling study obtained similar estimates for proportions of aerobic versus anaerobic degradation of 17% and 83% respectively[30].
The BTEX compounds have varying fates in the plume. Toluene and o-xylene degrade under methanogenic conditions near the oil body[41] while benzene and ethylbenzene do not degrade until reaching the iron reducing zone[42]. Since 1995, the position of the 50 µg/L benzene contour has remained stable at about 125 m downgradient and benzene concentrations near the source zone have dropped from almost 7 mg/L to 4 mg/L as the oil body concentrations of benzene have decreased[2]. These data indicate that natural attenuation under mainly iron-reducing conditions is effectively limiting the advance of the benzene plume.

About 60-80% of the reduced carbon in the north pool plume consists of nonvolatile dissolved organic carbon (NVDOC)[34][2]. Comparing concentrations in the same wells of NVDOC to total petroleum hydrocarbons in the diesel range (TPHd) shows that the TPHd values are less than one-third of the NVDOC values (Figure 5). The difference is due to losses of polar compounds during extraction into an organic solvent and retention on a gas chromatograph column. Concentrations of NVDOC were measured on filtered samples by acidifying and purging to removed inorganic carbon followed by combustion[2]. Often the term DOC is used for this analysis for surface water samples, but for hydrocarbon-contaminated sites the modifier nonvolatile clarifies that the volatile hydrocarbons present in the water samples have been removed during the purging process. Thus, NVDOC represents the total dissolved organic carbon (TDOC) minus the volatile organic carbon (VOC)[34]. The NVDOC in the contaminant plume is mainly composed of partial transformation products of the hydrocarbons in the oil[43].
Concentrations of NVDOC in the aquifer increase from <2 mg/L in the clean background to 30-50 mg/L under the north oil pool. The pool of compounds comprising the NVDOC is progressively oxidized as it migrates downgradient in the aquifer leading to a 90% decrease in concentration by 150-200 m downgradient. However, the position of the 5 mg/L NVDOC contour expanded downgradient from 125 m in 1988 to over 200 m in 2010[2]. During biodegradation, there is change in character from aliphatic, low oxygen-to-carbon ratio (O/C) compounds under the oil to more aromatic, high O/C compounds downgradient[32]. Ultrahigh resolution mass spectrometry analyses show that over 10,000 compounds are present in the NVDOC. Thus, assessing whether the mixture poses a human health or environmental hazard is hampered by the high number of individual compounds and low concentrations of any single compound. An assessment of the entire mixture of nonvolatile compounds extracted from wells located within 100 m of the north oil pool showed increased activity of toxicologically relevant transcriptional pathways and human nuclear receptors in human liver cells[4]. The measured effects decrease as NVDOC concentrations and properties change as a result of continued biotransformation during migration away from the source zone. As stated by McGuire, et al., 2018: “effects observed at the molecular level do not necessarily indicate adverse outcomes at the organismal level”[4]. The potential health effects of polar hydrocarbon oxidation products is an active and controversial area of research (see for example the publication by Zemo et al., 2013[44] and comments on that publication by Brewer and Blumberg[45]. For additional discussion see the ITRC TPH guidance document available online[46].
Ongoing Studies
The site continues to be an active research facility for understanding natural attenuation processes as well as developing methods for measuring remediation timeframes and plume toxicity. Ongoing process studies cover the properties of hydrophobic soils in the spray zone, causes of variations in NSZD rates, natural attenuation of secondary water quality impacts from iron and arsenic in the plume, and the toxicity and composition changes of the NVDOC. Methods studies cover remediation of hydrophobic soils, use of electrical and magnetic signals to monitor biodegradation rates, and geophysical techniques for mapping plume discharge to the lake. Outside researchers are welcome at the site and should check the policy webpage for more information.
Summary
A USGS research study of an August 1979 crude oil pipeline spill near Bemidji, Minnesota, continues to provide valuable results almost 40 years after the spill[3]. Analyses of oil samples indicate that 18-31% of the oil mass in the source zone had been lost by 2010 to natural processes of volatilization, dissolution, and biodegradation. Methods developed at the site for estimating NSZD rates include surface carbon dioxide efflux measurement and monitoring of increased subsurface temperatures.
In the groundwater plume, the most important natural attenuation processes are anaerobic biodegradation coupled to iron reduction, followed by fermentation and methanogenesis. The downgradient front of the benzene plume has been stable since 1995 and peak groundwater benzene concentrations are dropping as benzene concentrations decrease in the oil. Most of the dissolved organic carbon transported from the source zone in the groundwater plume consists of partial transformation products of the oil compounds (also known as polar metabolites). Biotransformation in the aquifer decreases concentrations of these compounds, but a refractory fraction of about 10% discharges to a small lake 335 m from the source.
Ongoing studies are assessing the toxicity of the partial transformation products, testing remedial strategies for hydrophobic oil-coated soils, and developing geophysical techniques for monitoring NSZD and surface water discharge of the groundwater plume.
References
- ^ 1.0 1.1 1.2 1.3 Essaid, H.I., Bekins, B.A., Herkelrath, W.N. and Delin, G.N., 2011. Crude oil at the Bemidji site: 25 years of monitoring, modeling, and understanding. Groundwater, 49(5), pp.706-726. doi: 10.1111/j.1745-6584.2009.00654.x
- ^ 2.0 2.1 2.2 2.3 2.4 Bekins, B.A., Cozzarelli, I.M., Erickson, M.L., Steenson, R.A. and Thorn, K.A., 2016. Crude oil metabolites in groundwater at two spill sites. Groundwater, 54(5), pp.681-691. doi: 10.1111/gwat.12419
- ^ 3.0 3.1 U.S. Geological Survey, 2017. Crude Oil Contamination in a Shallow Outwash Aquifer - Bemidji, Minnesota
- ^ 4.0 4.1 4.2 4.3 McGuire, J.T., Cozzarelli, I.M., Bekins, B.A., Link, H. and Martinović-Weigelt, D., 2018. Toxicity Assessment of Groundwater Contaminated by Petroleum Hydrocarbons at a Well-Characterized, Aged, Crude Oil Release Site. Environmental Science & Technology, 52(21), pp.12172-12178. doi: 10.1021/acs.est.8b03657
- ^ Delin, G.N. and Herkelrath, W.N., 2014. Effects of a Dual‐Pump Crude‐Oil Recovery System, Bemidji, Minnesota, USA. Groundwater Monitoring & Remediation, 34(1), pp.57-67. doi: 10.1111/gwmr.12040
- ^ Dillard, L.A., Essaid, H.I. and Herkelrath, W.N., 1997. Multiphase flow modeling of a crude‐oil spill site with a bimodal permeability distribution. Water Resources Research, 33(7), pp.1617-1632. doi: 10.1029/97WR00857
- ^ 7.0 7.1 Bennett, P.C., Siegel, D.E., Baedecker, M.J. and Hult, M.F., 1993. Crude oil in a shallow sand and gravel aquifer—I. Hydrogeology and inorganic geochemistry. Applied Geochemistry, 8(6), pp.529-549. doi: 10.1016/0883-2927(93)90012-6
- ^ 8.0 8.1 8.2 8.3 8.4 8.5 8.6 8.7 Crystal Ng, G.H., Bekins, B.A., Cozzarelli, I.M., Baedecker, M.J., Bennett, P.C., Amos, R.T. and Herkelrath, W.N., 2015. Reactive transport modeling of geochemical controls on secondary water quality impacts at a crude oil spill site near Bemidji, MN. Water Resources Research. 51(6), pp. 4156-4183. doi:10.1002/2015WR016964
- ^ 9.0 9.1 9.2 Sihota, N.J., Trost, J.J., Bekins, B.A., Berg, A., Delin, G.N., Mason, B., Warren, E. and Mayer, K.U., 2016. Seasonal variability in vadose zone biodegradation at a crude oil pipeline rupture site. Vadose Zone Journal, 15(5). doi: 10.2136/vzj2015.09.0125
- ^ 10.0 10.1 Garg, S., Newell, C.J., Kulkarni, P.R., King, D.C., Adamson, D.T., Renno, M.I. and Sale, T., 2017. Overview of natural source zone depletion: processes, controlling factors, and composition change. Groundwater Monitoring & Remediation, 37(3), pp.62-81. doi:10.1111/gwmr.12219
- ^ 11.0 11.1 Bekins, B.A., Hostettler, F.D., Herkelrath, W.N., Delin, G.N., Warren, E. and Essaid, H.I., 2005. Progression of methanogenic degradation of crude oil in the subsurface. Environmental Geosciences, 12(2), pp.139-152. doi: 10.1306/eg.11160404036
- ^ Beaver, C.L., Williams, A.E., Atekwana, E.A., Mewafy, F.M., Abdel Aal, G., Slater, L.D. and Rossbach, S., 2016. Microbial communities associated with zones of elevated magnetic susceptibility in hydrocarbon-contaminated sediments. Geomicrobiology Journal, 33(5), pp.441-452. doi: 10.1080/01490451.2015.1049676
- ^ Bekins, B.A., Godsy, E.M. and Warren, E., 1999. Distribution of microbial physiologic types in an aquifer contaminated by crude oil. Microbial Ecology, 37(4), pp.263-275. doi: 10.1007/s002489900149
- ^ Amos, R.T., Mayer, K.U., Bekins, B.A., Delin, G.N. and Williams, R.L., 2005. Use of dissolved and vapor‐phase gases to investigate methanogenic degradation of petroleum hydrocarbon contamination in the subsurface. Water Resources Research, 41(2). doi: 10.1029/2004WR003433
- ^ 15.0 15.1 15.2 Sihota, N.J., Singurindy, O. and Mayer, K.U., 2010. CO2-efflux measurements for evaluating source zone natural attenuation rates in a petroleum hydrocarbon contaminated aquifer. Environmental Science & Technology, 45(2), pp.482-488. doi: 0.1021/es1032585
- ^ Hult, M.F., Grabbe, R.R. and Ragone, S.E., 1985. Distribution of gases and hydrocarbon vapors in the unsaturated zone. In US Geological Survey Program on Toxic Waste—Groundwater Contamination—Proceedings of the Second Technical Meeting (pp. 21-25). Report.pdf
- ^ 17.0 17.1 Chaplin, B.P., Delin, G.N., Baker, R.J. and Lahvis, M.A., 2002. Long-term evolution of biodegradation and volatilization rates in a crude oil-contaminated aquifer. Bioremediation Journal 6(3), pp. 237-255 doi: 10.1080/10889860290777594
- ^ 18.0 18.1 18.2 18.3 Baedecker, M.J., Eganhouse, R.P., Bekins, B.A. and Delin, G.N., 2011. Loss of volatile hydrocarbons from an LNAPL oil source. Journal of Contaminant Hydrology, 126(3-4), pp.140-152. doi: 10.1016/j.jconhyd.2011.06.006
- ^ 19.0 19.1 19.2 19.3 Baedecker, M.J., Eganhouse, R.P., Qi, H., Cozzarelli, I.M., Trost, J.J. and Bekins, B.A., 2018. Weathering of oil in a surficial aquifer. Groundwater, 56(5), pp.797-809. doi: 10.1111/gwat.12619
- ^ 20.0 20.1 20.2 Molins, S., Mayer, K.U., Amos, R.T. and Bekins, B.A., 2010. Vadose zone attenuation of organic compounds at a crude oil spill site—Interactions between biogeochemical reactions and multicomponent gas transport. Journal of Contaminant Hydrology, 112(1-4), pp.15-29. doi: 10.1016/j.jconhyd.2009.09.002
- ^ Landon, M.K., 1993. Investigation of mass loss based on evolution of composition and physical properties of spilled crude oil contaminating a shallow outwash aquifer (Doctoral dissertation, University of Minnesota)
- ^ Herkelrath, W.N., 1999, March. Impacts of remediation at the Bemidji oil spill site. In US Geological Survey Toxic Substances Hydrology Program - Proceedings of the Technical Meeting, Charleston, South Carolina (Vol. 3, pp. 195-200). Report.pdf
- ^ Sihota, N.J. and Mayer, K.U., 2012. Characterizing vadose zone hydrocarbon biodegradation using carbon dioxide effluxes, isotopes, and reactive transport modeling. Vadose Zone Journal, 11(4). doi: 10.2136/vzj2011.0204
- ^ Warren, E. and Bekins, B.A., 2018. Relative contributions of microbial and infrastructure heat at a crude oil-contaminated site. Journal of Contaminant Hydrology, 211, pp.94-103. doi: 10.1016/j.jconhyd.2018.03.011
- ^ 25.0 25.1 Ng, G.H.C., Bekins, B.A., Cozzarelli, I.M., Baedecker, M.J., Bennett, P.C. and Amos, R.T., 2014. A mass balance approach to investigating geochemical controls on secondary water quality impacts at a crude oil spill site near Bemidji, MN. Journal of Contaminant Hydrology, 164, pp.1-15.doi: 10.1016/j.jconhyd.2014.04.006
- ^ Anderson, R.T. and Lovley, D.R., 2000. Biogeochemistry: hexadecane decay by methanogenesis. Nature, 404(6779), pp.722-823 doi: 10.1038/35008145
- ^ Bekins B., Baedecker, M.J., Egahouse, R.P., Herkelrath, W.N., 2011. Long-term natural attenuation of crude oil in the subsurface. In: GQ10: Groundwater quality management in a rapidly changing world: Proceedings of the 7th International Groundwater Quality Conference, Schirmer, M.; Hoehn, E.; Vogt, T., Editors. IAH: Zurich, Switzerland. Report.pdf
- ^ Edwards, E.A. and Grbić-Galić, D., 1992. Complete mineralization of benzene by aquifer microorganisms under strictly anaerobic conditions. Appl. Environ. Microbiol., 58(8), pp.2663-2666. ISSN: 0099-2240; Online ISSN: 1098-5336.
- ^ National Research Council (NRC). 2000. Natural Attenuation for Groundwater Remediation. Washington, DC: The National Academies Press. doi: 10.17226/9792
- ^ 30.0 30.1 Essaid, H.I., Cozzarelli, I.M., Eganhouse, R.P., Herkelrath, W.N., Bekins, B.A. and Delin, G.N., 2003. Inverse modeling of BTEX dissolution and biodegradation at the Bemidji, MN crude-oil spill site. Journal of Contaminant Hydrology, 67(1-4), pp.269-299. doi: 10.1016/S0169-7722(03)00034-2
- ^ Mason, B. E., 2014. Groundwater and Surface Water Interactions Down-Gradient from a Decades-Old Crude Oil Spill, Beltrami County Minnesota. MS Thesis, University of Minnesota, Minneapolis, MN.
- ^ 32.0 32.1 Podgorski, D.C., Zito, P., McGuire, J.T., Martinovic-Weigelt, D., Cozzarelli, I.M., Bekins, B.A. and Spencer, R.G., 2018. Examining Natural Attenuation and Acute Toxicity of Petroleum-Derived Dissolved Organic Matter with Optical Spectroscopy. Environmental Science & Technology, 52(11), pp.6157-6166. doi: 10.1021/acs.est.8b00016
- ^ 33.0 33.1 Cozzarelli, I.M., Schreiber, M.E., Erickson, M.L. and Ziegler, B.A., 2016. Arsenic cycling in hydrocarbon plumes: secondary effects of natural attenuation. Groundwater, 54(1), pp.35-45. doi: 10.1111/gwat.12316
- ^ 34.0 34.1 34.2 Eganhouse, R.P., Baedecker, M.J., Cozzarelli, I.M., Aiken, G.R., Thorn, K.A. and Dorsey, T.F., 1993. Crude oil in a shallow sand and gravel aquifer—II. Organic geochemistry. Applied Geochemistry, 8(6), pp.551-567. doi: 10.1016/0883-2927(93)90013-7
- ^ Gutierrez-Neri, M., Ham, P.A.S., Schotting, R.J. and Lerner, D.N., 2009. Analytical modelling of fringe and core biodegradation in groundwater plumes. Journal of Contaminant Hydrology, 107(1-2), pp.1-9. doi: 10.1016/j.jconhyd.2009.02.007
- ^ Baedecker, M.J., Cozzarelli, I.M., Eganhouse, R.P., Siegel, D.I. and Bennett, P.C., 1993. Crude oil in a shallow sand and gravel aquifer-III. Biogeochemical reactions and mass balance modeling in anoxic groundwater. Applied Geochemistry, 8(6), pp.569-586. doi: 10.1016/0883-2927(93)90014-8
- ^ Lovley, D.R., Baedecker, M.J., Lonergan, D.J., Cozzarelli, I.M., Phillips, E.J. and Siegel, D.I., 1989. Oxidation of aromatic contaminants coupled to microbial iron reduction. Nature, 339(6222), p.297. doi: 10.1038/339297a0
- ^ 38.0 38.1 38.2 Amos, R.T., Bekins, B.A., Cozzarelli, I.M., Voytek, M.A., Kirshtein, J.D., Jones, E.J.P. and Blowes, D.W., 2012. Evidence for iron‐mediated anaerobic methane oxidation in a crude oil‐contaminated aquifer. Geobiology, 10(6), pp.506-517. doi: 10.1111/j.1472-4669.2012.00341.x
- ^ Bekins, B.A., Cozzarelli, I.M., Godsy, E.M., Warren, E., Essaid, H.I. and Tuccillo, M.E., 2001. Progression of natural attenuation processes at a crude oil spill site: II. Controls on spatial distribution of microbial populations. Journal of Contaminant Hydrology, 53(3-4), pp.387-406. doi: 10.1016/S0169-7722(01)00175-9
- ^ Amos, R.T. and Mayer, K.U., 2006. Investigating the role of gas bubble formation and entrapment in contaminated aquifers: Reactive transport modelling. Journal of Contaminant Hydrology, 87(1-2), pp.123-154. doi: 10.1016/j.jconhyd.2006.04.008
- ^ Eganhouse, R.P., Dorsey, T.F., Phinney, C.S. and Westcott, A.M., 1996. Processes affecting the fate of monoaromatic hydrocarbons in an aquifer contaminated by crude oil. Environmental Science & Technology, 30(11), pp.3304-3312. doi: 10.1021/es960073b
- ^ Anderson, R.T., Rooney-Varga, J.N., Gaw, C.V. and Lovley, D.R., 1998. Anaerobic benzene oxidation in the Fe (III) reduction zone of petroleum-contaminated aquifers. Environmental Science & Technology, 32(9), pp.1222-1229. doi: 10.1021/es9704949
- ^ Thorn, K.A. and Aiken, G.R., 1998. Biodegradation of crude oil into nonvolatile organic acids in a contaminated aquifer near Bemidji, Minnesota. Organic Geochemistry, 29(4), pp.909-931. doi: 10.1016/S0146-6380(98)00167-3
- ^ Zemo, D.A., O'Reilly, K.T., Mohler, R.E., Tiwary, A.K., Magaw, R.I. and Synowiec, K.A., 2013. Nature and estimated human toxicity of polar metabolite mixtures in groundwater quantified as TPHd/DRO at biodegrading fuel release sites. Groundwater Monitoring & Remediation, 33(4), pp.44-56. doi: 10.1111/gwmr.12030
- ^ Brewer, R.C. and Hellmann‐Blumberg, U., 2014. Nature and estimated human toxicity of polar metabolite mixtures in groundwater quantified as TPHd/DRO at biodegrading fuel release sites. Groundwater Monitoring & Remediation, 34(2), pp.24-25. doi: 10.1111/gwmr.12053
- ^ Interstate Technology Regulatory Council (ITRC), 2018. TPH Risk Evaluation at Petroleum-Contaminated Sites